Very High Temperature Reactor (VHTR)
The Very High Temperature Reactor (VHTR), one of six Gen IV nuclear system candidates, is designed for electricity and heat cogeneration, and its high outlet temperature is ideal for hydrogen production and use in the chemical, oil, and iron industries. Drawing on operational experience from past or operating gas-cooled reactors in five countries, the VHTR features TRi-structural ISOtropic (TRISO) fuel, helium coolant, and low power density that supports passive decay heat removal. While the original approach for VHTR focused on very high outlet temperatures for hydrogen production, research and market assessments suggest that modest outlet temperatures (700-850°C) are sufficient and limit technical challenges associated with deployment. It also promises inherent safety, high thermal efficiency, process heat capability, low operational costs, and modular construction.
Presentation of the Very High Temperature Reactor System
Among the 6 candidates of the Gen IV nuclear systems in the technical roadmap of Gen IV International Forum (GIF), the Very High Temperature Reactor (VHTR) is primarily dedicated to the cogeneration of electricity and heat. Its high outlet temperature makes it attractive for hydrogen production as well as for the chemical, oil and iron industries.
The reference design considered by GIF utilizes the TRi-structural ISOtropic (TRISO) particles based fuel, helium coolant, as well as the dedicated core layout and lower power density to allow removal of decay heat through passive mechanisms.
The VHTR technology benefits from the operational feedback of 40 Gas Cooled Reactors (GCR, CO2 cooled) and 7 High Temperature Gas Reactors (HTGR, He cooled) gained in 5 different countries. The VHTR has potential for inherent safety, high thermal efficiency, process heat application capability, low operation and maintenance costs, and modular construction.
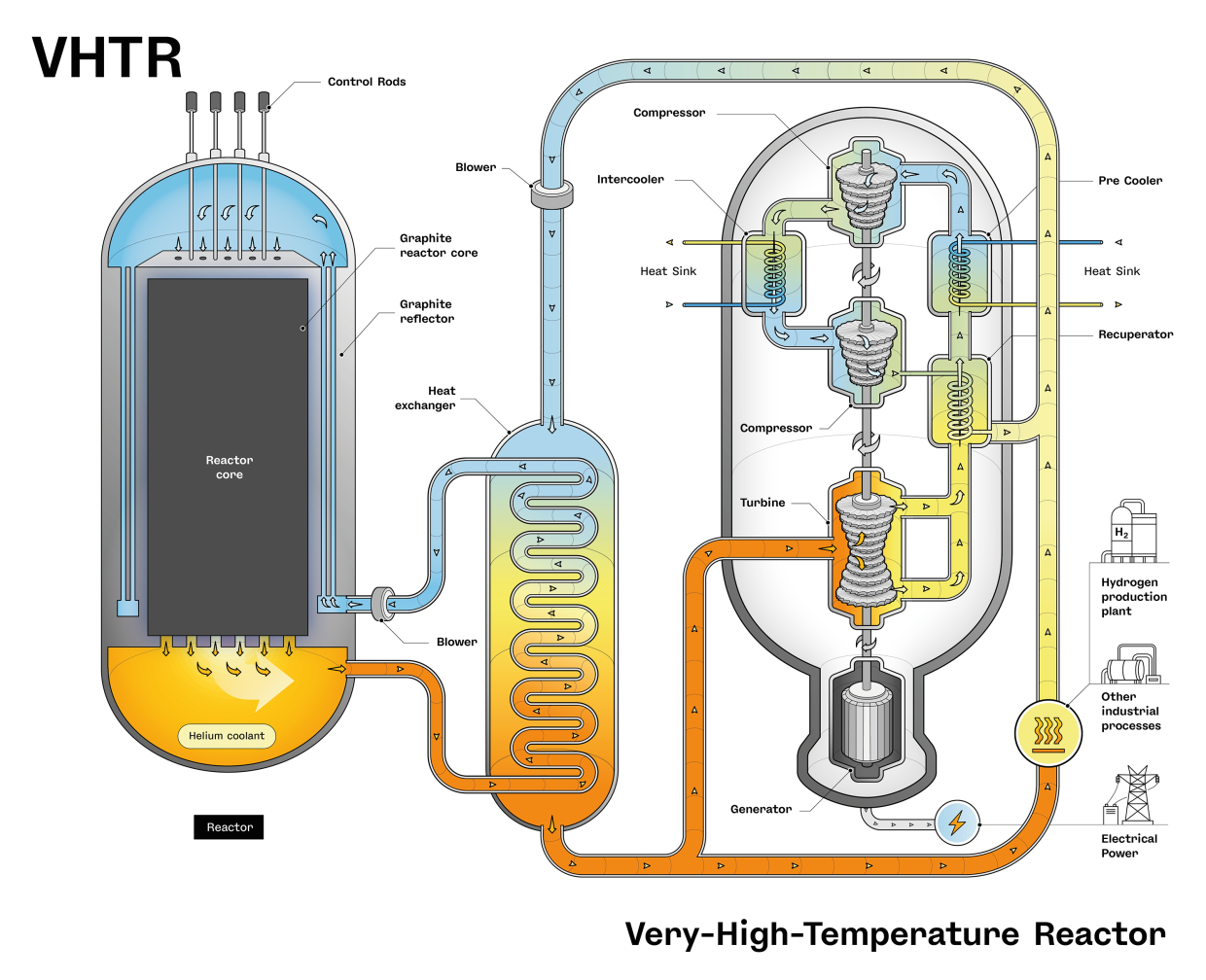
Attributes of the VHTR
The VHTR is a next step in the evolutionary development of high-temperature gas-cooled reactors (HTGRs). It is a graphite-moderated, helium-cooled reactor with thermal neutron spectrum. It can supply nuclear heat and electricity over a range of core outlet temperatures between 700 and 950°C, and potentially more than 1 000°C in the future.
The base elements of VHTR fuel are TRISO particles, each smaller than a millimeter in diameter. Surrounding this kernel are layers of carbon and silicon carbide, providing stable containment for fission products at temperatures of 1600°C or higher. These particles can be either integrated in cylindrical compacts integrated in blocks resembling hexagonal 'prisms' made of graphite (prismatic-block type core) or encased in silicon carbide within graphite in pebbles of about 60mm in diameter (pebble-bed type core).
Although the shapes of the fuel compounds for these two configurations are different, the technical basis for both is the same: TRISO coated particles fuel in a graphite matrix, fully ceramic (graphite) core structure, helium coolant, and low power density, to achieve high outlet temperature and the retention of fission production inside the coated particle under normal operation condition and accident condition.
As mentioned before, to allow passive decay heat removal, each core options should be kept below the following power limits:
- Below 625 MWth for prismatic-block type
- Below 250 MWth for pebble bed type
A VHTR can be designed to use alternative and symbiotic fuel cycles such as U-Pu, MOX or Th-U.
For electricity generation, a direct cycle with a helium gas turbine system directly placed in the primary coolant loop, or, at the lower end of the outlet temperature range, an indirect cycle with a steam generator and a conventional Rankine cycle can be used. Today, the direct Brayton power conversion cycle is being pursued less aggressively in favour of an indirect Rankine cycle because of its lower technological risk and increased flexibility in terms of working fluid and mission of the reactor (electricity, process heat, and co-generation).
For nuclear heat applications such as process heat for refineries, petrochemistry, metallurgy and hydrogen production, the heat application process is generally coupled with the reactor through an intermediate heat exchanger (IHX), in what is often called an indirect cycle.
VHTR Reactor Parameters | Reference Value |
---|---|
Spectrum | Thermal |
Core Outlet Temperature range | Graphite |
Coolant | 700–950°C with the goal to reach above 1000° temperatures at a later stage. |
Primary pressure | He Gas |
Power Range | 10 MWth to 842 MWth |
Fuel | Uranium containing (enriched in U-235) TRISO based pebbles or compacts. A VHTR can be designed to use alternative and symbiotic fuel cycles such as U-Pu, MOX or Th-U. |
How do Very High Temperature Reactors meet Generation IV Criteria?
Resource utilization
Increased fuel Burnup and improved fuel utilization: the VHTR enables to reach high fuel burnup levels (approaching 200 GWd/t) that are about 3 to 4 times higher than thdxcse burnup rates of contemporary Light Water Reactor (LWR) fuels. This allows for better utilization of the fissile material contained in the fuel before taking it out of the reactor. This feature also enhances the economic parameters of VHTR.
Waste Minimisation & Management
In relation to the higher fuel utilization VHTRs have the potential to reduce the amount of radioactive waste per unit of power produced through achieving higher burnup, or through reprocessing fuel. A VHTR can also be designed to use alternative and symbiotic fuel cycles such as U-Pu, MOX or Th-U.
In case of open cycle with no reprocessing the TRISO based used fuel is very stable, reliable at confining radioactive elements and the residual heat from radioactivity does not require active cooling.
Use in areas with scarce water resources
Designs using direct gas energy conversion system, eliminating water and steam systems, and enabling economical dry cooling tower allow for for inland and remote reactor siting without abundant cooling water. Air cooling is also possible with a defendable efficiency penalty.
Lower construction and operating costs
Once the technology mature VHTR should have lower construction costs, notably because of the absence of thick metallic pressure vessels and massive containment buildings.
High Efficiency
VHTRs operate at high temperatures, enabling high thermal efficiency in electricity generation. This can lead to a more cost-effective conversion of heat to electricity compared to current Generation III nuclear reactors.
Pebble bed small modular reactors of the HTR-PM utilize steam power conversion system reach 40% net efficiency with a 750°C reactor outlet temperature.
Other concepts of VHTRs using prismatic type core and a direct gas turbine cycle, like Japan's proposed GTHTR300, are expected to reach thermal efficiencies of 46%–51% at 850–950°C reactor outlet temperatures.
The increased fuel burnup also allows to reduce fuel costs and improve the economics of the VHTR reactors.
Process Heat Applications
VHTRs can provide high-temperature process heat in addition to electricity generation. This versatility allows VHTR to be employed in various industrial applications, such as hydrogen production (including potentially innovative hydrogen production methods such as Sulfur Iodine cycle that runs at 830°C) or other processes requiring very elevated temperatures including in the petrochemical industry, ammonia production and fertilizer production.
Type of fuel
TRISO particle-based fuels are highly resistant to neutron irradiation, corrosion, oxidation, and high temperatures. The triple-coated layers of each particle act as a self-contained system, guaranteeing the retention of fission products under various reactor conditions. Fission product retention has been demonstrated up to 1600°C.
The coated particle fuel form is the primary barrier to fission product release. The coatings, in particular the silicon carbide layer, effectively retain the bulk of the radionuclide inventory under all anticipated operating and accident conditions. The fuel performance envelope was largely established in the first generation HTGR programs in the US and Germany which specified limits on power density, temperature, fluence, burnup, and packing fraction. Recent fuel fabrication and qualification efforts have further improved the performance with respect to particle failures and radionuclide release. The performance of TRISO fuel is demonstrated through qualification testing (irradiations and post-accident heating) under conditions that fully envelope those of the reactor under all anticipated operating and accident conditions.
Management of waste
The reprocessing of TRISO particles fuel is still being investigated. Yet the TRISO particles themselves, notably before of the characteristics mentioned just above are great at encapsulating radioactive elements in a very stable envelope.
Radiation Protection
Internal radiation levels in the VHTR plant are generally lower than those in LWR because of the low circulating activity in the coolant, mainly in the form of graphite dust on which some radionuclides may be adsorbed or otherwise carried.
Other intrinsic safety features and very long grace periods:
The VHTR design makes use of the inherent safety features of a graphite-moderated core cooled by helium. The graphite possesses substantial thermal inertia, and the helium coolant is a single-phase, inert substance with no reactivity effects.
The VHTR core has a low power density, a high heat capacity, and a slender core shape (large height-to-diameter ratio). The transients resulting from Loss of Forced Cooling (LOFC) events develop and occur over tens or hundreds of hours; a very long ‘grace period’ relative to LWRs. If forced cooling of the VHTR core is lost, decay heat is transferred naturally via conduction and radiation from the core, through the outer reflector and reactor pressure vessel (RPV), and on to the ultimate heat sink. For VHTR, the removal of decay heat does not require active cooling with helium.
Proliferation Resistance:
The key areas of strengths of the VHTR concept are its robust fuel form, with fissile material strongly diluted in carbonaceous material, high burnup and the use of the once-through LEU fuel cycle, which all make VHTR fuel unattractive for proliferation purposes.
To obtain a significant quantity of either indirect-use U-235 from LEU or direct-use plutonium, one must process metric tons and tens of cubic meter quantities of carbon encasing fuel using either grind-leach or burn-leach of electrolysis in nitric acid, which is very challenging.
Plutonium and U-233 in VHTR spent fuel are difficult to extract and divert due to high radioactivity, encapsulation, and material dilution, making them unattractive for non-state actors seeking nuclear material.
Physical Protection:
Due to the intrinsic safety features of the VHTR design and its fuel (low power density, very stable and temperature resistant fuel form, containment of radionuclides within TRISO fuel, strong negative temperature coefficient, etc.) VHTRs are a difficult target for acts of sabotage that would have the goal to cause a nuclear accident on site (loss of cooling or loss of control over reactivity of the reactor) or a radiological one (dispersal of radioactive materials).
Benefits and Applications of Very High Temperature Reactors
While the original approach for VHTR at the start of the Generation IV program focused on very high outlet temperatures and hydrogen production, current market assessments have indicated that electricity production and industrial processes based on high temperature steam that require modest outlet temperatures (700-850°C) have the greatest potential for application in the next decade while also reducing the technical challenges associated with higher outlet temperatures. As a result, the focus has moved from higher outlet temperature designs such as in GT-MHR and PBMR reactors to lower outlet temperature designs such as HTR-PM in China and the NGNP in the US. These experiences should nevertheless pave the way for higher output temperature capable materials in the future.
Most of the benefits and advantages of VHTR designs have been listed in the above description of how those reactors meet the Generation IV criteria. Yet the potential, opened by very high outlet temperatures of VHTRs, for an expanded range of applications of nuclear heat cannot be understated.
Main Challenges for the Deployment of VHTR
Demonstrating the viability of the VHTR core requires solving several significant technical challenges. Fuels and materials must be developed that:
- permit an increase of the core-outlet temperatures from around 800°C to more than 1 000°C for the entire plant lifetime;
- permit the maximum fuel temperature under accident conditions to reach levels approaching 1 800°C;
- permit maximum fuel burnup of 150-200 GWd/tHM;
- avoid power peaking and temperature gradients in the core, as well as hot streaks in the coolant gas;
- limit structural degradation from air or water ingress.
An important amount of work is needed regarding the use of the reactor heat for industrial processes and the coupling of a nuclear plant with other industrial facilities.
Process-specific R&D gaps need to be filled to adapt the chemical process and the nuclear heat source to each other regarding temperatures, power levels and operational pressures. Heating of chemical reactors by helium is a departure from current industrial practice and needs specific R&D and demonstration. The development of an intermediate heat exchanger, ducts, valves and associated heat transfer fluid is needed to deliver process heat to many of the chemical processes. The viability of using nuclear process heat to produce hydrogen needs further study. Any contamination of the product will have to be avoided. Development of heat exchangers, coolant gas ducts and valves will be necessary for isolation of the nuclear island from the production facilities. This is especially the case for isotopes like tritium, which can easily permeate metallic barriers at high temperatures. Over the past two decades, significant advances have been made in the key technologies necessary to deploy a VHTR. Further R&D is needed, and GIF is actively participating to deploy VHTR.
How is GIF working to solve those
In it’s 2014 Technology Roadmap GIF identified the following areas of R&D as priorities for the VHTR system:
- Fuels and Materials
- Reactor Systems
- Balance of Plant
- Fuel Cycle
- Economics
- Safety Objectives
To know more please consult the 2014 GIF Technology Roadmap.
GIF has established System Steering Committees (SSC) to implement the research and development (R&D) for each Generation IV Reactor Concept, with participation from GIF Members interested in contributing to collaborative R&D efforts on the said system. Each SSC plans and integrates R&D projects contributing to the development of a system.
Each SSC is governed by a System Arrangement (SA), provisional SSCs are created under a Memorandum of Understanding (MoU).
Several projects can be carried within an SSC, each project is government by a Project Arrangement (PA) and managed by a Project Management Board (PMB).
The GIF VHTR SSC was established in 2006. To effectively support the development of the VHTR technology/system and to overcome jointly the challenges identified on the path to do so, the GIF coordinates several international R&D activities related to VHTR system. They are now arranged by the VHTR SSC Signatories into four joint R&D projects.
VHTR Projects History - Past projects
The VHTR technology benefits from the operational feedback of 40 Gas Cooled Reactors (GCR, CO2 cooled) and 9 High Temperature Gas Reactors (HTGR, He cooled) gained in 7 different countries (China, France, Germany, Japan, Spain, UK, US).
The High-Temperature Gas-Cooled Reactor (HTGR) has a history spanning over 50 years, marked by successes and challenges. The initial experimental plants included a 20 MWe unit in the UK and a 15 MWe unit in Germany, operational in 1966. The German project, intended to burn thorium, faced political and technical obstacles, leading to its abandonment in 1988.
In the U.S., two commercial power-producing HTR plants were constructed. The first, a 200 MWth/115MWth experimental unit at Peach Bottom, operated from 1967 to 1974, providing valuable insights. These findings contributed to the development of a larger 330 MWe plant at Fort St Vrain, active from 1979 to 1989. While this reactor demonstrated the technical feasibility of HTRs, further development was needed to establish their economic viability.
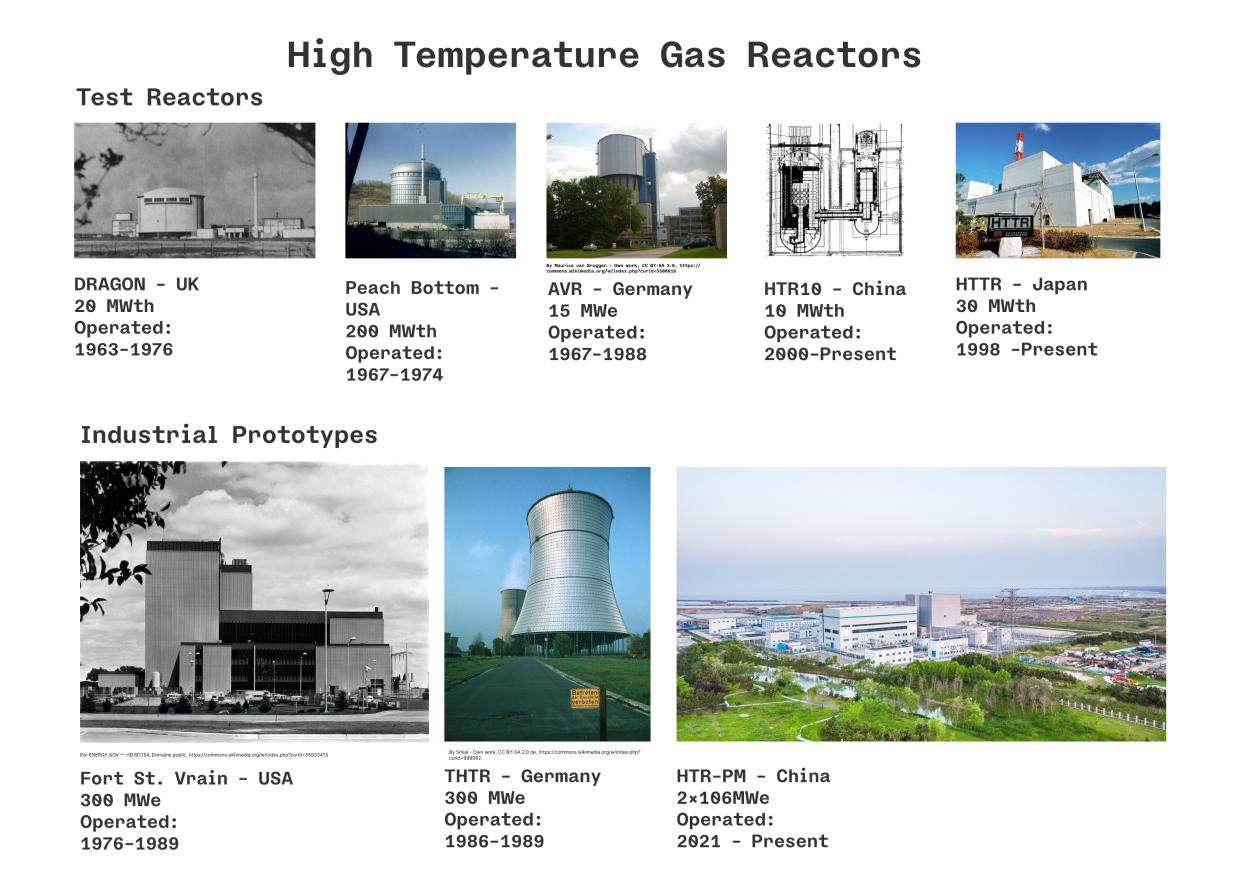
Project/Program | Country | Power Rating | Timeframe | Notes |
---|---|---|---|---|
High-Temperature Gas-Cooled Reactor (HTGR) | University of Chicago, US | N/A | 1944 - 1947 | Initial proposal of HTGR |
Dragon Reactor | United Kingdom | 21.5 MWth | 1965 - 1976 | Exploring use of TRISO fuel |
Peach Bottom Unit 1 Reactor | United States | 200 or 115 MWth | 1966 - 1974 | First HTGR to produce electricity |
AVR Reactor | Germany | 46 MWth | 1967 - 1988 | Exploring use of carbide BISO, and TRISO particle fuel |
Fort St. Vrain Generating Station | United States | 842 MWth | 1979 - 1989 | Load-following power plant |
THTR-300 | Germany | 750 MWth | 1983 - 1989 | Prototype reactor using TRISO fuel |
HTR-10 | China | 10 MWth | First criticality in 2000 - Stopped in 2019 | Prototype reactor of pebble-bed HTGR |
VHTR Current Developments
Operating VHTR Reactors
Three VHTR/HTGRs plants are currently operating with the HTR-PM being a commercial scale nuclear power plant with two reactors feeding one turbine.
Project Name | Country | Power Rating | Expected Deployment Date | Notes |
---|---|---|---|---|
High-Temperature Engineering Test Reactor | Japan | 30 MWth | First criticality in 1998, operated 50 days in 2010, restarted in July 2021. | Outlet temperature of 950°C was reached at full thermal power in 2004 for the first time in the world. One of the current focuses is demonstrating elements related to coupling with H2 production. |
HTR-PM (Shidao Bay 1) | China | 250 MWth/reactor 200MWe net output for the whole plant with two reactors feeding one turbo-generator. | Criticality 2021 / Commercial operation December 2023 | Scaled-up version of HTR-10 |
Status of VHTR designs
Project/Program | Country | Power Rating | Expected Deployment Date | Notes |
---|---|---|---|---|
GTHTR300C | Japan Atomic Energy Agency, Japan | 600 MWth 274 MWe | Conceptual | 45~50% thermal efficiency |
PMBR | Pebble Bed Modular Reactor (Pty) Limited / Eskom, South Africa | 400 MWth / 200MWe | On Hold | International Cooperation design team, aimed for industrial application |
Prismatic HTR | General Atomics, US | 350 MWth 150 MWe | Under Design | TRISO particles, assembling in 2 steps. |
SC-HTGR | Framatome, France/USA | 625 MWth 272 MWe | Not known | Based on HTR-MODUL |
U-Battery | Urenco, UK-Germany-The Netherlands | 10 MWth 4 MWe | Cancelled in 2023 | Based on Dragon reactor/Fort St. Vrain reactor |
USNC MMR | Ultra Safe Nuclear Corporation , US | 10~45 MWth 3.5~15 MWe | Pre-licensing steps carried out in Canada (VDR 1 done, VDR 2 ongoing, application for a license to prepare the site) | Micro reactors, Ceramic core design, Heat storage to allow for flexibility of output without having to cycle the reactor |
Holos-Quad HTR | Hologen, US Military | 22 MWth 10 MWe | Under development with ANL | Transportable in a 40-foot shipping container |
Adams Engine | Adams Atomic Engines | 10 MWe | Design shelved | Liquid nitrogen coolant |
HTMR-100 | Steenkampskraal Thorium Limited, South Africa | 100 MWth 35 MWe | Conceptual | Very strong negative temperature coefficient |
GT-MHR | Cooperation between the USA and Russia | 600 MWth 285 MWth | On hold before construction | Multi-layer ceramic coating fuel |
MIGHTR - Modular Integrated Gas High Temperature Reactor | Boston Atomics, US | 20-600MWth | In development since 1984 |
GIF VHTR Related Publications
GIF has produced several reports and conducted analysis on VHTR Systems produced by cross cutting methodological working groups (Risk and Safety WG, Proliferation Resistance & Physical Protection Working Group). GIF's annual reports, technology roadmap and R&D Outlooks provide more information on the progress made by GIF's VHTR System Steering Committee and Project Management Boards.
GIF VHTR Related Webinars
References
2023, Handbook of Generation IV Nuclear Reactors, Second Edition, Dr. Igor L. Pioro, Handbook of Generation IV Nuclear Reactors | ScienceDirect
International Atomic Energy Agency Advanced Reactors Information System (ARIS) Online Database
News related to the VHTR System
December 2023, China's demonstration HTR-PM enters commercial operation - https://www.world-nuclear-news.org/Articles/Chinese-HTR-PM-Demo-begins-commercial-operation -
August 2023, Fuelling high temperature SMRs - https://www.neimagazine.com/features/featurefuelling-high-temperature-smrs-11110962/ -
May 2021, UK / Nuclear Company Announces HTGR Collaboration With Japan - https://www.nucnet.org/news/nuclear-company-announced-htgr-collaboration-with-japan-5-4-2021 ,
August 2020, USNC, Korean companies to develop micro modular reactors - https://www.world-nuclear-news.org/Articles/USNC-Korean-companies-to-develop-micro-modular-rea,
November 2015, VHTR cooling system performance verified - https://www.world-nuclear-news.org/Articles/VHTR-cooling-system-performance-verified